A Mechanism for Shallow Methane Hydrate Dissociation
Does Freshwater Runoff in the Arctic change Ocean Circulation to Unlock Methane Hydrate in the Deep Ocean?
This post covers: Identification of possible mechanism which could eventually release vast quantities of shallow Methane Hydrate into the ocean and atmosphere, from current observation and modelling.
“Temperature increase of the bottom waters can lead to the thawing of the frozen bottom sediments and the release of additional amount of methane from gas hydrates.” Source
A key challenge in assessing the impact of dissociating gas hydrates on global atmospheric methane is the lack of a technique able to distinguish between methane recently released from gas hydrates and methane emitted from leaky thermogenic reservoirs, shallow sediments (some newly thawed), coal beds, and other sources. Source
At high latitudes, methane hydrates also occur at depths of ~225 m or greater in and beneath continuous permafrost. Source
Ocean deep-water formation is sensitive to changes in surface buoyancy and momentum fluxes. Changes such as temperature decrease or salinity increase lead to an increase in density relative to underlying waters and causes the water mass to sink (Pond and Pickard, 1983) Source
For the shallow ESAS, fall convection is particularly important in late September to early October (freeze-up period) when the probability of convection penetrating down to the seafloor can reach 40–50% (Kulakov et al., 2003)
2012: The Arctic region has received considerable attention due to its sensitivity to the changing climate. Of particular concern is the rapid decline of the summer sea ice extent, which this year even may approach another record low since satellite observations begun 1979. The sea ice in the Arctic forms at the top of the ‘halocline’, a 200–300 m thick layer of low salinity seawater capping the Arctic Ocean. The low salinity of the halocline layer is reflects the high freshwater to the Arctic Ocean. The halocline is also very cold, close to freezing point of seawater near -2°C, protecting the sea ice from the deeper laying warmer and more saline Atlantic Water Layer that flows into the Arctic Ocean from the North Atlantic.
The new study published as a Letter in Nature Geoscience shows that the warm intermediate Atlantic Layer was displaced far downward in the glacial Arctic Ocean, resulting in a substantial warming at depths between 1000 and 2500 m. Based on a conceptual oceanographic model, the researchers propose a mechanism for the subsurface warming of the glacial Arctic Ocean: A reduced influx of freshwater to the Arctic Ocean acted to deepen the halocline and push the warm Atlantic Layer downward. Based on their results, the researchers conclude that the Arctic Ocean has a previously unrecognized high sensitivity to changes of the freshwater input over multiple timescales, which is manifested in large temperature excursions of the intermediate water layers. Source
Is it possible that due to freshwater inflow(River runoff with high sedimentation rates) into the Arctic Ocean and/or because of the Albedo Flip Effect(Ice loss), warmer water mass formation occurred at the bottom of shallow marine sediments? Apparently observation show bottom water warmth and is in agreement with modelling(Shakhova).
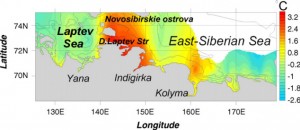
The following study from 2006 explores pathways of methane from sediments into the atmosphere
Methane release and coastal environment in the East Siberian Arctic shelf, N. Shakhova, I. Semiletov, Journal of Marine Systems 66 (2007) 227–243
We believe that a few additional points concerning mechanisms of methane ventilation to the atmosphere should be taken into consideration.
First, the estimate assumes a diffusion mechanism for methane transport to the sea surface, but the most powerful mechanism for methane transport in shallow shelf areas is bubbling or ebullition (Chanton et al., 1989). Ebullition transports methane directly to the atmosphere, bypassing the mediating effects of an oxygenated sediment–water interface and water column. A common misunderstanding is that dissolved methane must exceed the solubility of the gas in water (roughly 1 mM at 20 °C and P CH4 = 1 atm) for bubbles to form. In fact, bubbles can form at methane concentrations well below saturation (Chanton et al., 1989).
The proportion of methane bubbles that survives passing through the water column to reach the atmosphere generally depends on water depth and bubble size. It was shown by Semiletov et al. (1996)that from a depth of 10 m, bubbles with radius of 0.1 cm reach the air–water interface in 62 s losing only 15.1% of their methane. As mean depth within the study area was 123 m in 2003 and 13.1 m in 2004, we can assume that ignoring ebullition is a major gap in our estimates.
Second, the Arctic Ocean has a natural trap for the gas bubbles — sea ice. During the freeze-up period gas bubbles are accumulated within ice structures in direct proportion to surface water saturation, and in inverse proportion to the speed of freeze-up (Zubov, 1938). Bubbles observed within the fast ice can reach up to 10 cm in diameter, and can completely deform the bottom of the fast ice. It is documented in the literature that after ice within Dmitri Laptev Strait was demolished using explosives (for the purpose of improving navigation) a flame of burning methane was seen (Zhigarev, 1997). We measured concentrations of methane beneath the ice (November, 1994) equal to 20,000 nM (Semiletov et al., 2004). When the ice begins to break up, methane accumulated beneath the ice may be abruptly released into the atmosphere.
Third, ventilation of methane through the annual ice is possible because of its brine content. It was shown that methane was enriched in the air 2 m above the ice up to 4 ppm (Kelley and Gosink, 1979). In summer time (June) during the ice break period they measured concentration of methane in surface seawater increased up to 107 nM. A number of recent studies have shown that sea ice is highly permeable to gases through numerous tiny channels (Gosink et al., 1976; Semiletov et al., 2004). In addition, more than 1% of shelf area consists of open polynyas (Kulakov et al., 2003) providing a pathway for methane to escape during the winter period.
Fourth, for the shallow ESAS, fall convection is particularly important in late September to early October (freeze-up period) when the probability of convection penetrating down to the seafloor can reach 40–50% (Kulakov et al., 2003). During the summer period the surface layer warmed up to 8.5 °C (Fig. 2); subsequent cooling during ice formation leads to vertical convection. Therefore, convective mixing may homogenize the concentration of dissolved methane in a body of water and increase super-saturation in the surface water following the significant release of methane into the atmosphere before ice formation is complete.
Fifth, it was mentioned above that the late summer period was much windier in 2004 than in 2003. Since the rate of gas exchange between the ocean surface and the atmosphere is a function of wind speed, this perturbation might have affected methane release substantially. Moreover, the changing of the relatively stable summer situation on the Arctic shelf to fall storm conditions appears to be a possible triggering mechanism for increasing the methane flux (Wanninkhof, 1992). In our estimates using long-term mean wind speed (6 m/s) instead of actual measured wind speed alters the resulting flux up to + 9 times. Source
Possible methane emissions from the East Siberian Arctic Seas
Global changes in the Arctic occur in the growth of average air temperatures, rapidly melting ice on land and sea, and underwater permafrost degradation. The extensive Arctic Shelf can play an important role in methane cycling because of the huge storage of organic matter buried in permafrost, which can be involved in the modern biogeochemical cycles under warming. The dissolved methane concentrations in the East Siberian Arctic Shelf water during summers of 2003 to 2008 show a widespread oversaturation in large spatial scales [1]. The horizontal and vertical methane distributions in the observational data indicate a sedimentary source which is likely associated with thawing of the underwater permafrost and release of gas from the shallow Arctic gas hydrate.
The analysis of the thermohaline characteristics of the East Siberia Shelf water in the model run showed the positive trend in the bottom temperature, which is in agreement with the observational data. Temperature increase of the bottom waters can lead to the thawing of the frozen bottom sediments and the release of additional amount of methane from gas hydrates. Assuming the increase in the gas permeability of the perennial frozen sediment caused by climate change, the numerical simulation of the dissolved methane transport from the bottom reservoirs in the shelf water was performed.
A three-dimensional mathematical model of the dissolved gas transport by the ocean currents with the parameterization of the oxidation process was used for the quantitative evaluation of the scale of a possible methane flux from the submarine sources. According to our numerical results obtained in the period from 2002 to 2010, the total methane emission in the eastern Arctic shelf waters can be estimated from 16 to 54 kilotons per year, which is two orders lower than the estimates given in [1]. Source
Results obtained for the sedimentation rate during the Holocene show that the highest values(>100 cm kyr−1) are restricted to the shelf of the Circum-Arctic realm and other shallow water areas, where marine GH are not stable. Source (Page 963)
Methane release from warming-induced hydrate dissociation in the West Svalbard continental margin: Timing, rates, and geological controls
K. E. Thatcher,G. K. Westbrook,S. Sarkar,T. A. Minshull
Source: Hundreds of plumes of methane bubbles, first observed in 2008, emanate from an area of the seabed off West Svalbard that has become 1°C warmer over the past 30 years. The distribution of the plumes, lying close to and upslope from the present upper limit of the methane hydrate stability zone, indicates that methane in the plumes could come from warming-induced hydrate dissociation, a process commonly invoked as contributing to rapid climate change.
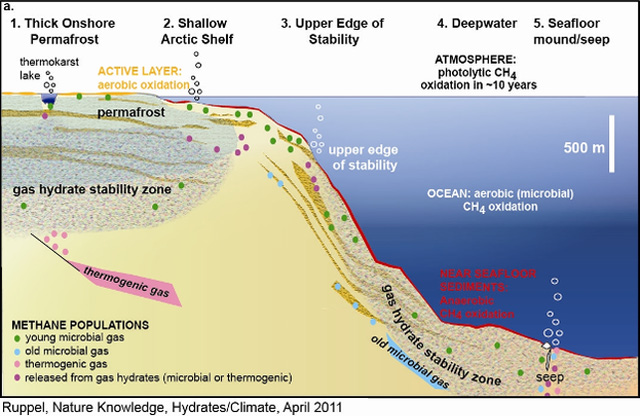
Figure 1a: Gas hydrate sectors and their response to climate change.
Source: Schematic cross-section from a high-latitude ocean margin (onshore permafrost and shallow offshore subsea permafrost) in Sectors 1 and 2 on the left, across a generic upper continental slope (Sector 3), and into a deepwater marine gas hydrate system (Sector 4) and an area of gas seeps on the right (Sector 5). The horizontal scale on Arctic Ocean margins may range from less than 102 to 103 km. GHSZ sediments usually have low saturations of methane hydrate, except in permeable sand layers shown here with coarser-grained texture. Ice-bonding within permafrost follows similar relationships. New microbial CH4(green) can be formed where labile organic carbon is available, including within the GHSZ, beneath lakes in permafrost areas, and in newly thawed sediments above subsea or terrestrial permafrost. Red zones at and below the seafloor denote anaerobic CH4 oxidation (coinciding with sulfate reduction), which occurs in zones that thin with increasing CH4 flux. The orange zone onshore denotes seasonal aerobic CH4 oxidation in the annually thawed active layer. CH4 oxidation associated with lakes in permafrost is not depicted. Methane, not necessarily derived from gas hydrates, is emitted directly to the atmosphere at ebullition sites in shallow lakes within permafrost and probably in open water on shallow Arctic shelves. Methane emitted at the seafloor at greater water depths is not likely to reach the atmosphere.
Extensive Methane Venting to the Atmosphere from Sediments of the East Siberian Arctic Shelf
Shakhova, Natalia; Semiletov, Igor; Salyuk, Anatoly; Yusupov, Vladimir; Kosmach, Denis; Gustafsson, Örjan
Source: Remobilization to the atmosphere of only a small fraction of the methane held in East Siberian Arctic Shelf (ESAS) sediments could trigger abrupt climate warming, yet it is believed that sub-sea permafrost acts as a lid to keep this shallow methane reservoir in place. Here, we show that more than 5000 at-sea observations of dissolved methane demonstrates that greater than 80% of ESAS bottom waters and greater than 50% of surface waters are supersaturated with methane regarding to the atmosphere. The current atmospheric venting flux, which is composed of a diffusive component and a gradual ebullition component, is on par with previous estimates of methane venting from the entire World Ocean. Leakage of methane through shallow ESAS waters needs to be considered in interactions between the biogeosphere and a warming Arctic climate.
Methane release from the East-Siberian Arctic Shelf and its connection with permafrost and hydrate destabilization: First results and potential future developments
Shakhova, N.; Semiletov, I.
Source: Until recently, the ESAS was not considered a CH4 source due to the supposed impermeability of sub-sea permafrost, which was thought to completely isolate the CH4 beneath from modern biogeochemical cycles. However, the ESAS represents an enormous potential CH4 source that could be responsive to ongoing global warming. Such response could occur in substantially shorter time than that of terrestrial Arctic ecosystems, because sub-sea permafrost has experienced long-lasting destabilization initiated by its inundation during the Holocene ocean transgression. ESAS permafrost stability and integrity is key to whether sequestered ancient carbon escapes as the potent greenhouse gas CH4. Recent data suggest the sub-sea permafrost is currently experiencing significant changes in its thermal regime. For example, our recent data obtained in the ESAS during the drilling expedition of 2011 showed no frozen sediments at all within the 53 m long drilling core at water temperatures varying from -0.6°C to -1.3°C. Unfrozen sediments provide multiple potential CH4 migration pathways. We suggest that open taliks have formed beneath the areas underlain or influenced by the nearby occurrence of fault zones, under paleo-valleys, and beneath thaw lakes submerged several thousand years ago during the ocean transgression. Temporary gas migration pathways might occur subsequent to seismic and tectonic activity in an area, due to sediment settlement and subsidence; hydrates could destabilize due to development of thermokarst-related features or ice-scouring.
Recently obtained geophysical data identified numerous gas seeps, mostly above prominent reflectors, and the ubiquitous occurrence of shallow gas-charged sediments containing numerous gas chimneys, underscoring the likelihood that the ability of sub-sea permafrost to capture CH4 released from the seabed is failing. Available data suggest the ESAS sub-sea permafrost is currently leaking a substantial amount of CH4. We propose that a few different types of CH4 exist, and are becoming involved in the modern carbon cycle due to permafrost destabilization in the ESAS: modern biogenic CH4 produced from ancient substrate, relatively old biogenic CH4 mobilized from hydrate deposits, and old thermogenic CH4 accumulated within seabed deposits. Isotopic data obtained by sampling CH4 in the water column and atmospheric CH4 in close proximity to the sea surface confirm the contribution from different sources, and demonstrate that the isotopic signature of CH4 from the ESAS can be used to create an interpretive plot for defining hydrates.
CH4 fluxes could occur as numerous weak seeps, as large areas of strong bubble plumes, or as sites where CH4 releases are flare- or torch-like and the emissions are non-gradual. Due to the shallow and oligotrophic nature of the ESAS, the majority of aqueous CH4 may avoid biological oxidation in the water column and escape to the atmosphere. Further investigations should be focused on quantifying the total CH4 pool of the ESAS, improving our understanding of the mechanisms responsible for sub-sea permafrost destabilization and gas migration pathways formation, and decreasing uncertainties regarding the current CH4 emission mode and its future alteration by progressing permafrost degradation.
References
- On the possible methane emissions from the East Siberian Arctic Seas
- Methane release from warming-induced hydrate dissociation in the West Svalbard continental margin: Timing, rates, and geological controls
- Estimation of the global inventory of methane hydrates in marine sediments using transfer functions
- Mass fractionation of noble gases in synthetic methane hydrate: Implications for naturally occurring gas hydrate dissociation
- Methane release from the East-Siberian Arctic Shelf and its connection with permafrost and hydrate destabilization: First results and potential future developments
- Deep Arctic Ocean warming during the last glacial cycle
- Methane Hydrates and Contemporary Climate Change
- Extensive Methane Venting to the Atmosphere from Sediments of the East Siberian Arctic Shelf
- On The Sensitivity Of Ocean Circulation To Arctic Freshwater Pulses During The Paleocene/Eocene Thermal Maximum
- Methane release and coastal environment in the East Siberian Arctic shelf, N. Shakhova, I. Semiletov, Journal of Marine Systems 66 (2007) 227–243
Further Reading
- Past extreme warming events linked to massive carbon release from thawing permafrost
- Using isotopes to understand methane formation, removal and transport in the East Siberian Arctic Shelf
- Tempo and scale of late Paleocene and early Eocene carbon isotope cycles:Implications for the origin of hyperthermals
About the Author: Chris Machens
