Estimating northern polar CH4 flux
A compilation of related science with some commentary.
Microbes in thawing permafrost: the unknown variable in the climate change equation
David E Graham, Matthew D Wallenstein, Tatiana A Vishnivetskaya, Mark P Waldrop,Tommy J Phelps, Susan M Pfiffner, Tullis C Onstott, Lyle G Whyte, Elizaveta M Rivkina,David A Gilichinsky, Dwayne A Elias, Rachel Mackelprang, Nathan C VerBerkmoes,Robert L Hettich, Dirk Wagner, Stan D Wullschleger and Janet K Jansson | The ISME Journal advance online publication, 17 November2011; doi:10.1038/ismej.2011.163
Considering that 25% of Earth’s terrestrial surface is underlain by permafrost (ground that has been continuously frozen for at least 2 years), our understanding of the diversity of microbial life in this extreme habitat is surprisingly limited. Taking into account the total mass of perennially frozen sediment (up to several hundred meters deep),permafrost contains a huge amount of buried, ancient organic carbon (Tarnocaiet al., 2009). In addition, permafrost is warming rapidly in response to global climate change (Romanovskyet al., 2010), potentially leading to widespread thaw and a larger, seasonally thawed soil active layer.
This concern has prompted the question: will permafrost thawing lead to the release of massive amounts of carbon dioxide (CO2) and methane (CH4) into the atmosphere?
This question can only be answered by understanding how the microbes residing in permafrost will respond to thaw, through processes such as respiration, fermentation, methanogenesis and CH4 oxidation (Schuuret al., 2009). Predicting future carbon fluxes is complicated by the diversity of permafrost environments, ranging from high mountains, southern boreal forests, frozen peatlands and Pleistocene ice complexes (yedoma) up to several hundred meters deep, which vary widely in soil composition, soil organic matter(SOM) quality, hydrology and thermal regimes.
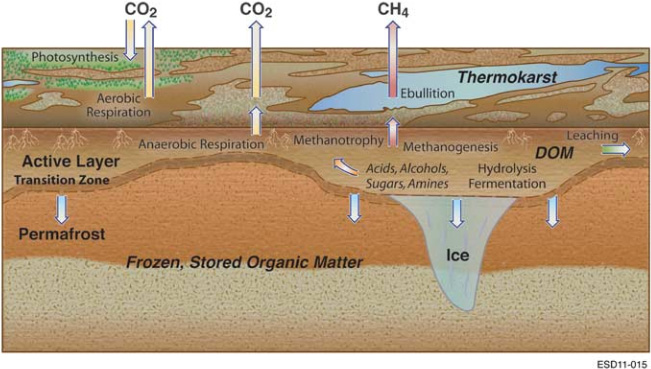
Fig. Key biological processes in the carbon cycle of permafrost environments. Permafrost thawing at the transition zone introduces previously unavailable organic matter into the expanded active layer of soil. Enzymatic hydrolysis decomposes complex organic matter into soluble substrates for microbial fermentation, producing a mixture of organic acids, alcohols and microbial biomass. Methanogenicarchaea convert acetate, methylated compounds or H2and CO2into CH4that can be released to the atmosphere through ebullition,diffusion or aerenchyma. Methanotrophs oxidize some of this CH4, converting it to CO2
Permafrost degradation can occur in many forms: thaw can progress downward from seasonally-thawed ‘active layer’ soils in warming climates or laterally because of changes in surfaceor groundwater flow paths (Grosseet al., 2011). Permafrost degradation can sometimes lead to dramatic changes in ecosystem structure and function, such as the formation of thermokarst bogs. Wildfires and other disturbances that remove vegetation and organic matter warm the ground, hastening permafrost degradation. The complexity of the Northern Arctic and Subarctic environments in terms of geology, vegetation, paleo history and climate, suggests that understanding the microbial ecology in permafrost regions will require numerous studies throughout the Pan-Arctic.
Frozen conditions in permafrost efficiently preserve biological material from DNA to wooly mammoths. Low water potential, reduced protein flexibility and enzyme activity, limited membrane fluidity, and ice nucleation and melting are all potentially lethal, so it was long assumed that microbes were either dead or dormant when frozen. However, high ionic strength within pore water can depress the freezing point and preserve cell viability. Recent experiments demonstrated that permafrost microorganisms remain active at extremely low temperatures (Vishnivetskayaet al., 2006; Gilichinsky and Rivkina, 2011): indigenous bacteria incorporated 14C-labeled acetate into lipids down to -20°C, many isolates showed psychroactive growth at -2.5°C and acetotrophic methanogenesis continued between 5°C and -17°C. Thus, warming could induce SOM decomposition even before permafrost thaws completely.
Microbial activity at low temperatures could transform complex organic compounds to soluble metabolites and gases, including the greenhouse gases (GHG): CO2, CH4 and N2O. Microbial transformations of carbon and nitrogen compounds in the soil active layer can in turn affect plant productivity and community composition, changing animal habitats and affecting human land use. Although these gas fluxes and landscape changes can be directly measured, we need more detailed, mechanistic models that integrate geochemical and structural parameters with microbial processes to explain and predict future changes in permafrost regions. In order to accurately predict the vulnerability of permafrost carbon to decomposition and the resulting fluxes of GHG products, we should understand how Arctic microbial communities respond to permafrost thawing. In addition to an increase in the rate of organic matter decomposition because of warming, there will most likely be shifts in the microbial community composition and in the relative consumption of different organic constituents.
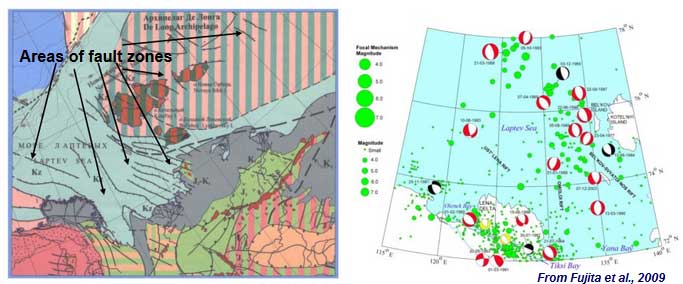
Geodynamics and sedimentary
Enhanced active fault zone characteristic is likely to create larger pulses of methane hydrate during short periods in time and space, because of the higher magnitude and possibility of shifting seafloor topographical features which disrupt the sub-sea permafrost layer. Observed seismicity can help to indicate potential hot spots.
CH4 increase is observed within the vicinity of river runoff flux increases significantly and possibly in the region of active fault zones when seismic moderates cryospheric features through abrupt force. If development occurs, seafloor release might develop permeable pathway venting through subsea permafrost, which i guess are rather gradual with isolated larger pulses from talik channeling.
The ESAS is tectonically and seismically active area, seismic events include endogenous (self organizing) seismicity. [2]
Shakhova et al.[2010] further highlighs the ESAS region:
Increase in river runoff to the ESAS is significant, noticeable in winter. Propagation of warm fresh waters is increasing and occurs laterally and top-down and causes further warming of the sub-sea permafrost in shallower parts of the ESAS. Source: Shakhova et al. (2010)
Methanogenesis
The ESAS provides favorable conditions for methanogenesis and accumulation of natural hydrocarbons
- The highest rates of carbon rain to the bottom provides required amount of organic carbon to be buried in the seabed; Annual sediment accumulation is about 10×10 ^12 g C yr-^1, which approximately equals the amount of sediment accumulated over the entire pelagic zone of the World Ocean.
- Stable conditions of the continental margin lead to formation of very thick sedimentary basins (up to 20 km thick), which provide temperature and pressure conditions required for methane production
- Geological conditions are favorable for formation of all types of seabed deposits: extensive coal beds, natural gas and oil fields; hydrate accumulations within and out of gas hydrate stability zone (GHSZ)
- Upward moving geofluids provide transport of hydrocarbons to the areas of gas hydrate stability zone (GHSZ). Source: Shakhova et al. (2010)
Mechanism of combined talik formation(geothermal heat + thermokarst)
Data (2003-2005) from the ESAS showed extreme surface water CH4 supersaturation (up to 22,000% saturation), implying high sea-to-air fluxes. [1]
Pathways
What is known about mechanisms of permafrost destabilization associated with formation of gas migration pathways?
There are two basic mechanisms of permafrost destabilization:
- 1) Natural process of permafrost equilibration (equilibrium balancing) with its warmer environment determines talik formation (a gradual process), this process is faster in fault zones.
- 2) Natural process of tectonic and seismic activity in the region (non-gradual process) causes permafrost breaks; this process is occurring wherever such events take place. Source: Shakhova et al.[2010]
Methane emissions from the ESAS depend on occurrence of gas migration pathways, developing through permafrost body (open taliks; permafrost breaks, discontinuity in sediment layers, fault zones, sediment settlement and adjustment etc.); this determines non-gradual emission mode. [2]
Estimates normally assume a diffusion mechanism for methane transport to the sea surface, the most powerful mechanism for methane transport in shallow shelf areas is bubbling or ebullition (Chanton et al., 1989). Ebullition transports methane directly to the atmosphere, bypassing the mediating effects of an oxygenated sediment–water interface and water column. A common misunderstanding is that dissolved methane must exceed the solubility of the gas in water (roughly 1 mM at 20 °C and P CH4 = 1 atm) for bubbles to form. In fact, bubbles can form at methane concentrations well below saturation (Chanton et al., 1989).[3]
Greenhouse Gas Origin
ESAS shallow ebullition pathways, land and sea based permafrost thaw initiated decomposition feedbacks – increased respiration rates, possibly enhanced methanogenesis within thermokarst formation (likely where methanogens dominate methanotrophs) all heat trapping gas sources which reach the atmosphere directly add up to the overall radiative forcing or indirectly – when the condition is favorable enough through increased N2O production. A 2011 study found:
Archaea in Arctic Thermokarst Lake Sediments
Assays of enrichment cultures incubated at 2 and 10 °C with substrates used in the main pathways for methanogenesis have produced positive growth and CH4 production results. Most cultures produced CH4 from carbon dioxide (CO2) reduction with hydrogen (H2), although methanol and acetate were also utilized as methanogenic substrates by a few cultures. From the experiments conducted to date we conclude that there is a great diversity of Archaea inhabiting these Thermokarst lakes, which include, in part, a methanogenic community. These findings suggest that the archaeal community in lake sediments of the Northern Slope of Alaska may be more relevant to estimates of methane release from the Arctic than previously thought. Our work will set the grounds to further the understanding of the effect of temperature increases on microbial activities that directly affect the greenhouse gas inventory, and it will expand the census of psychrophiles that thrive in permafrost environments. Link
Strangelove Ocean
Ocean hypoxia trends in coastal regions are likely stimulated from factors like higher rates of N2O production (S. W. A. Naqvi et al. 2010), in situ from stratification(Guillaume Borrel et al. 2012)[6] and dissolved methane hydrate in the shallow ESAS region and temperature dependent microbial community changes based on metabolic rates. (C. Knoblauch et al. 1999)[5] gases which doesn’t reach the atmospheric layer, or from biogenic origin (sub-sea permafrost layer) or because of erosion rates (ESAS erosion rate 80m per year) or from river particulate organic carbon discharge – which literally feeds methanogens. The increased CO2 availability from biogeochemical cycling, decreases the ocean PH and also leads to ocean acidification.[7]
Natural and human-induced hypoxia and consequences for coastalareas: synthesis and future development
J. Zhang, D. Gilbert, A. J. Gooday, L. Levin, S. W. A. Naqvi, J. J. Middelburg, M. Scranton, W. Ekau,A. Pẽna, B. Dewitte, T. Oguz, P. M. S. Monteiro, E. Urban, N. N. Rabalais, V. Ittekkot, W. M. Kemp,O. Ulloa, R. Elmgren, E. Escobar-Briones, and A. K. Van der Plas | 2010 | Source
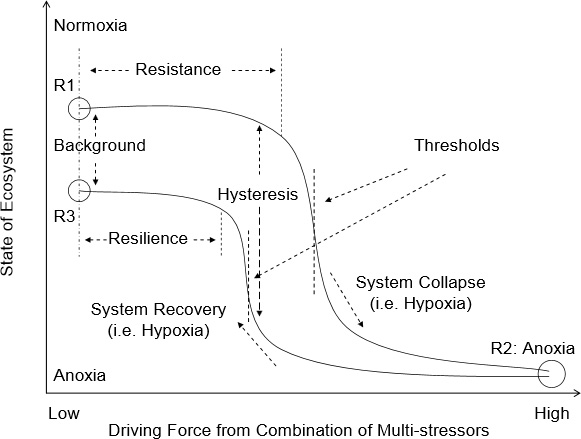
Hypoxia has become a world-wide phenomenon in the global coastal ocean and causes a deterioration of the structure and function of ecosystems. The present study provides an overview of the major aspects of coastal hypoxia in different biogeochemical provinces, including estuaries, coastal waters, upwelling areas, fjords and semi-enclosed basins, with various external forcings, ecosystem responses, feedbacks and potential impact on the sustainability of the fishery and economics. The obvious external forcings include freshwater runoff and other factors contributing to stratification, organic matter and nutrient loadings, as well as exchange between coastal and open ocean water masses.
Their different interactions set up mechanisms that drive the system towards hypoxia. Coastal systems also vary in their relative susceptibility to hypoxia depending on their physical and geographic settings. It is understood that coastal hypoxia has a profound impact on the sustainability of ecosystems, which can be seen, for example, by the change in the food-web structure and system function; other influences include compression and loss of habitat, as well as changes in organism life cycles and reproduction. In most cases, the ecosystem responds to the low dissolved oxygen in non-linear ways with pronounced feedbacks to other compartments of the Earth System, including those that affect human society.
Our knowledge and previous experiences illustrate that there is a need to develop new observational tools and models to support integrated research of biogeochemical dynamics and ecosystem behavior that will improve confidence in remediation management strategies for coastal hypoxia.
Methane-driven oceanic eruptions and mass extinctions
Gregory Ryskin | Department of Chemical Engineering, Northwestern University, Evanston, Illinois 60208, USA | 2003 | Source
Methane, CH4, is continuously produced beneath the ocean floor. Most of this methane is consumed by archaea and bacteria in the upper layer of sediments; the rest escapes into the overlying water column as bubbles. (For present purposes, it is immaterial whether some part of this methane flux results from dissociation of methane hydrates.) In a well-oxygenated(or anoxic but sulfate rich) water column, dissolved methane, as well as the sinking organic matter from the surface waters, is oxidized by microbes. But unlike the rising bubbles of methane or the sinking particles of organic matter, which can traverse the entire water column in a few days, oxygen molecules or sulfate ions can be delivered to the water column only by fluid flow (or diffusion, which is very slow). In stagnant water masses, therefore, the oxidation potential of the water column will be eventually overwhelmed by the flux of sinking organic matter, plus the flux of methane bubbles from the seafloor.
There is strong evidence in the geological record that dissolved methane may escape oxidation in the water column (Thomas et al., 2002). Under such conditions, methane that dissolves in the water column will accumulate in it as time proceeds.
Evidence of such accumulation exists both in direct measurements and in the geological record. The only long-term series of the former, the Cariaco Basin, shows that methane concentration in the deep waters of the basin is increasing with time, roughly linearly (Figs. 6d and 7 of Scranton et al., 2001). The micro fossil carbon isotope data from the Santa Barbara Basin sediment core show that high levels of dissolved methane were present periodically in the water column (Ken-nett et al., 2000). This has been confirmed by a microbial-biomarker study (Hinrichs et al., 2003), and is likely to be representative of oceanic regions prone to stagnation and anoxia, such as silled basins or deep-water masses isolated by topography and Taylor columns. (Such regions are likely to have sizes below the resolution of ocean circulation models used in biogeochemical studies; e.g., Hotinski et al.[2001].)
The time scale of the meridional overturning circulation,;103yr, has little relevance for the water masses that do not participate in it; these can only be mixed by turbulence resulting from breaking of the internal gravity waves (ultimately due to tides and winds). The corresponding vertical (diapycnal) turbulent diffusivity K is typically;10^-5 m^2 s^-1, and can be as low as 0.5 x 10^-6 m^2 s^-1(Gregg et al.,2003); the latter translates into K = 16 m^2 yr^-1. This means that it may take a million years for turbulent mixing to penetrate vertically through (Kt)^1/2 4km (t being time). (The tracer-based estimates ofthe age of water masses are controversial [Wunsch, 2002] and are not used here.) In stagnant water masses, accumulation of dissolved methane may thus continue for very long times. Assuming that dissolved methane can accumulate to high concentrations.
Future Arctic Ocean primary productivity from CMIP5 simulations: Uncertain outcome, but consistent mechanisms
Martin Vancoppenolle,Laurent Bopp,Gurvan Madec,John Dunne,Tatiana Ilyina,Paul R. Halloran,Nadja Steiner | Article first published online: 1 JUL 2013 DOI: 10.1002/gbc.20055
Net Arctic Ocean primary production (PP) is expected to increase over this century, due to less perennial sea ice and more available light, but could decrease depending on changes in nitrate (NO3) supply. Here Coupled Model Intercomparison Project Phase 5 simulations performed with 11 Earth System Models are analyzed in terms of PP, surface NO3, and sea ice coverage over 1900–2100. Whereas the mean model simulates reasonably well Arctic-integrated PP (511 TgC/yr, 1998–2005) and projects a mild 58 TgC/yr increase by 2080–2099 for the strongest climate change scenario, models do not agree on the sign of future PP change. However, similar mechanisms operate in all models. The perennial ice loss-driven increase in PP is in most models NO3-limited. The Arctic surface NO3 is decreasing over the 21st century (−2.3 ± 1 mmol/m3), associated with shoaling mixed layer and with decreasing NO3 in the nearby North Atlantic and Pacific waters. However, the intermodel spread in the degree of NO3 limitation is initially high, resulting from >1000 year spin-up simulations. This initial NO3 spread, combined with the trend, causes a large variation in the timing of oligotrophy onset—which directly controls the sign of future PP change. Virtually all models agree in the open ocean zones on more spatially integrated PP and less PP per unit area. The source of model uncertainty is located in the sea ice zone, where a subtle balance between light and nutrient limitations determines the PP change. Hence, it is argued that reducing uncertainty on present Arctic NO3 in the sea ice zone would render Arctic PP projections much more consistent. SourceHave sudden large releases of methane from geological reservoirs occurred since the Last Glacial Maximum, and could such releases occur again?
Euan G. Nisbet | 2002 | Published 15 April 2002 doi: 10.1098/rsta.2001.0958 Phil. Trans. R. Soc. Lond. A 15 April 2002 vol. 360 no. 1793 581-607 | Source
Methane emissions from geological reservoirs may have played a major role in the sudden events terminating glaciation, both at the start of the Bølling/Allerød and also at the end of the Younger Dryas. These reservoirs include Arctic methane hydrates and also methane hydrate stored in offshore marine sediments in tropical and temperate latitudes. Emissions from hydrate stores may have resonated with tropical wetland emissions, each reinforcing the other. Because methane is such a powerful greenhouse gas, much smaller emissions of methane, compared with carbon dioxide, are required in order to have the same short–term impact by climate forcing. The methane–linked hypothesis has much geological support from sea–floor evidence of emission. However, Greenland ice–core records have been interpreted as showing methane as a consequential factor, rather than the leader, of change. This interpretation can be challenged on the grounds that temperature gradients in Greenland ice record local changes and local timing of a step–like shift in weather fronts, while methane concentrations record changes on a hemispheric and global scale. There are large remaining hydrate reservoirs in the Arctic and in shelf sediments globally, and there is substantial risk of further emissions.Marine hypoxia/anoxia as a source of CH4 and N2O
S. W. A. Naqvi, H. W. Bange, L. Faŕıas, P. M. S. Monteiro, M. I. Scranton, and J. Zhang | Published: 12 July 2010
From 2009: When CH4 accumulates in O2-deficient sub-surface waters, methanotrophic activity severely restricts its diffusive efflux to the atmosphere. As a result, an intensification or expansion of coastal O2-deficient zones will probably not drastically change the present status where emission from the ocean as a whole forms an insignificant term in the atmospheric CH4 budget. The situation is different for N2O, the production of which is greatly enhanced in low-O2 waters, and although it is lost through denitrification in most suboxic and anoxic environments, the peripheries of such environments offer most suitable conditions for its production, with the exception of enclosed anoxic basins. Most O2-deficient systems serve as strong net sources of N2O to the atmosphere. This is especially true for coastal upwelling regions with shallow O2-deficient zones where a dramatic increase in N2O production often occurs in rapidly denitrifying waters. Nitrous oxide emissions from these zones are globally significant, and so their ongoing intensification and expansion is likely to lead to a significant increase in N2O emission from the ocean. However, a meaningful quantitative prediction of this increase is not possible at present because of continuing uncertainties concerning the formative pathways to N2O aswell as insufficient data from key coastal regions. SourceRelated Microbial biogeochemistry of coastal upwelling regimes in a changing ocean (August 2013)
The new sea ice regime favors more ocean circulation, which will likely trigger-enhance gradual thaw modes in the hydrosphere (sub-sea bottom warming with increased perturbations) in the rest of the shallow ESAS.
Combined changes are large and pronounced from the entire Arctic Circle. Over time carbon equivalent and methane greenhouse gas concentrations will just add up significantly. The cryospheric setup today is unique, the rate of change – forcings, acceleration with release events and tipping thresholds, the radiative efficacy, stratospheric ozone destruction and there is more. Ultimately a lot depends on ecosystem management and net emissions from today.
Forcing
Strong atmospheric chemistry feedback to climate warming from Arctic methane emissions
Ivar S. A. Isaksen, Michael Gauss, Gunnar Myhre, Katey M. Walter Anthony, Carolyn Ruppel | Article first published online: 20 APR 2011 | DOI: 10.1029/2010GB003845
The magnitude and feedbacks of future methane release from the Arctic region are unknown. Despite limited documentation of potential future releases associated with thawing permafrost and degassing methane hydrates, the large potential for future methane releases calls for improved understanding of the interaction of a changing climate with processes in the Arctic and chemical feedbacks in the atmosphere. Here we apply a “state of the art” atmospheric chemistry transport model to show that large emissions of CH4 would likely have an unexpectedly large impact on the chemical composition of the atmosphere and on radiative forcing (RF). The indirect contribution to RF of additional methane emission is particularly important. It is shown that if global methane emissions were to increase by factors of 2.5 and 5.2 above current emissions, the indirect contributions to RF would be about 250% and 400%, respectively, of the RF that can be attributed to directly emitted methane alone. Assuming several hypothetical scenarios of CH4 release associated with permafrost thaw, shallow marine hydrate degassing, and submarine landslides, we find a strong positive feedback on RF through atmospheric chemistry. In particular, the impact of CH4 is enhanced through increase of its lifetime, and of atmospheric abundances of ozone, stratospheric water vapor, and CO2 as a result of atmospheric chemical processes. Despite uncertainties in emission scenarios, our results provide a better understanding of the feedbacks in the atmospheric chemistry that would amplify climate warming. LinkAtmospheric composition, radiative forcing, and climate change as a consequence of a massive methane release from gas hydrates
Gavin A. Schmidt,Drew T. Shindell | 31 JAN 2003 | DOI: 10.1029/2002PA000757 | Source
The massive perturbation to global climate and the carbon cycle during the Paleocene/Eocene Thermal Maximum (PETM) (approx. 55.5 Ma) may have been forced by a catastrophic release of methane gas (CH4) from hydrate deposits on the continental slope. We investigate whether reported PETM paleotemperature and paleo-CO2 proxies are consistent with this hypothesis by considering the impact of large increases in CH4 emissions to the atmosphere. Significant effects on atmospheric chemistry and CH4 lifetime are seen for a range of plausible emission rates (1500 Gt carbon over 500–20,000 years). The resulting peak anomalous radiative forcing is 1.5–13.3 W/m2 depending on the emission scenario. The scenarios most closely matched to the PETM carbon isotope excursion have peak forcing of around 3 W/m2, which translates to peak temperature changes as a function of latitude that are a reasonable match to derived estimates. High CH4 levels and enhanced stratospheric water vapor amounts persist for as long as do the emissions and are responsible for more of the peak radiative forcing than CO2 levels, although results are sensitive to the background climate state and base CH4 concentration
The effect for atmospheric CH4 accumulation in the atmosphere, with increased lifetime:
Reaction between methane and hydroxyl removes both species from the atmosphere. As the concentration of hydroxyl reduces, the rate of methane removal will slow down. Increasing atmospheric methane concentrations removes hydroxyl from the atmosphere and so slows its own removal.
•Because the hydroxyl radical – the cleanser of the atmosphere – is capable of reacting with many species, methane is not the only influence on its concentration. Sources of hydroxyl (mainly ozone) are roughly constant, but it may be removed from the atmosphere by reactions with carbon monoxide (CO), nitrogen dioxide (NO2), hydroperoxide (HO2) and volatile organic compounds. In particular, the reaction between carbon monoxide and hydroxyl proceeds very rapidly, so carbon monoxide scavenges hydroxyl from the atmosphere. Increased anthropogenic emissions of carbon monoxide (from transport), coupled with the further carbon monoxide produced from oxidation of methane, can cause significant hydroxyl concentration reductions and so slow the rate of methane removal.
As the rate of methane removal slows, its lifetime in the atmosphere and therefore its GWP will increase. Methane will become a more potent greenhouse gas over time if hydroxyl concentrations continue to decrease. In terms of policy, it is more effective to reduce methane emissions now while hydroxyl concentrations remain relatively high.
Delaying action to reduce methane emissions, until a time when hydroxyl concentrations are lower, will result in the emitted methane being more potent. Furthermore, successful methane emission reductions, preferably accompanied by lower anthropogenic carbon monoxide emissions, could result in an increase in hydroxyl concentrations and a subsequent lowering of the GWP of future methane emissions. This beneficial positive feedback loop is a further reason for encouraging methane emissions reduction in the short term Link
David Archer created an online model for methane estimates, based on Hansen’s factor of 1.4 efficacy,
An online model of methane in the atmosphere (January 2012)
[Response:Yes, depletion of OH is what allows the lifetime to go up with methane load. The indirect radiative effects are accounted for with Hansen’s factor of 1.4 efficacy. David] LinkClimate Sensitivity Estimated From Earth’s Climate History (2012)
James E. Hansen and Makiko Sato | NASA Goddard Institute for Space Studies and Columbia University Earth Institute, New York
Earth’s climate history potentially can yield accurate assessment of climate sensitivity. Imprecise knowledge of glacial-to-interglacial global temperature change is the biggest obstacle to accurate assessment of the fast-feedback climate sensitivity,which is the sensitivity that most immediately affects humanity. Our best estimate for the fast-feedback climate sensitivity from Holocene initial conditions is 3± 0.5°C for 4W/m2 CO2 forcing (68% probability). Slow feedbacks, including ice sheet disintegration and release of greenhouse gases(GHGs) by the climate system, generally amplify total Earth system climate sensitivity. Slow feedbacks make Earth system climate sensitivity highly dependent on the initial climate state and on the magnitude and sign of the climate forcing, because of thresholds (tipping points) in the slow feedbacks. It is difficult to assess the speed at which slow feedbacks will become important in the future, because of the absence in paleoclimate history of any positive (warming) forcing rivaling the speed at which the human-caused forcing is growing. Link
References
[1] Degrading offshore permafrost as a source of methane (2011)
Joye Research Group and Drs. Igor Semiletov and Natasha Shakohova Source
[2] Methane release from the East Siberian Arctic Shelf and the Potential for Abrupt Climate Change (2010)
N. Shakhova, Igor Semiletov | University of Alaska, Fairbanks, International Arctic Research Centre, USA | Source
[3] Methane release and coastal environment in the East Siberian Arctic shelf
N. Shakhova, I. Semiletov, Journal of Marine Systems 66 (2007) 227–243 | Source
[4] Methane-driven oceanic eruptions and mass extinctions (2003)
Gregory Ryskin | Department of Chemical Engineering, Northwestern University | Source
[5] Community size and metabolic rates of psychrophilic sulfate-reducing bacteria in Arctic marine sediments
C Knoblauch, B B Jørgensen, J Harder | 1999 | Source
[6] Stratification of Archaea in the Deep Sediments of a Freshwater Meromictic Lake: Vertical Shift from Methanogenic to Uncultured Archaeal Lineages
Guillaume Borrel, Anne-Catherine Lehours, Olivier Crouzet, Didier Jézéquel, Karl Rockne, Amélie Kulczak, Emilie Duffaud, Keith Joblin, Gérard Fonty | 2012 | Source
[7] A “Strangelove” ocean in the earliest Tertiary
K. J. Hsü and J. A. McKenzie | GEOPHYSICAL MONOGRAPH SERIES, VOL. 32, PP. 487-492, 1985 | Source
A decrease of up to 3°/oo in the δ13 C values of planktic skeletons has been systematically observed across the Cretaceous/Tertiary boundary; the benthic skeletons show no corresponding changes. We interpret this decrease as a manifestation of the elimination of the surface-to-bottom carbon isotope gradient in ocean waters at a time when carbon fractionation by a photosynthesis-respiration mechanism became ineffective. A concurrent release of excess CO2 from a nearly barren ocean to the atmosphere could have caused global warming.
Further reading
An examination of the influence of thermokarst activity on arctic lake sediment methanogenesis
Methane production in aerobic oligotrophic surface water in thecentral Arctic Ocean
Methane production may occur as a rapid response to envi-ronmental perturbations during the shift from a phytoplank-ton bloom to an oligotrophic system, induced by a switchin the utilization of phosphate and methylated compounds.Hence, methane production in aerobic surface water is di-rectly linked to the N, P and C cycles. Recent change in theArctic has altered seasonal ice coverage and density stratifi-cation of surface water, which may have profound effects onthese biogeochemical cycles. Thus, feedback effects on cy-cling pathways of the climatically relevant biogases methaneand DMS are likely, with DMSP catabolism in high latitudespossible contributing to a warming effect on the earth’s cli-mate through production of the greenhouse gas, methane.
Calcium isotope constraints on the end-Permian mass extinction
Explained: Radiative forcing
Community size and metabolic rates of psychrophilic sulfate-reducing bacteria in Arctic marine sediments
Popular Posts
FinalCut Pro License Fundraiser
About the Author: Chris Machens
